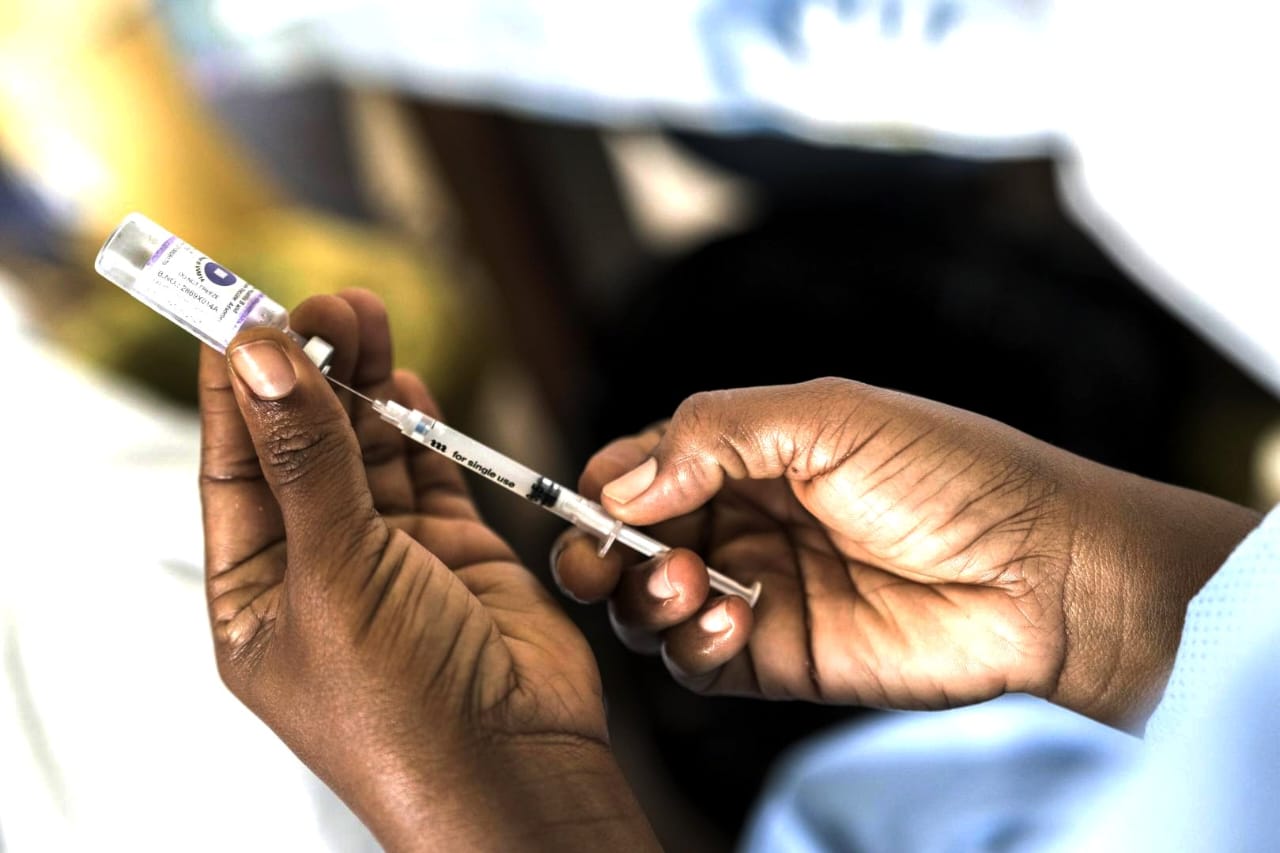
Influenza has long been a viral adversary that keeps scientists, clinicians, and public health officials on their toes year after year. Every season, millions around the globe receive flu shots to protect themselves from the strains predicted to dominate that winter. Yet despite decades of vaccination efforts, up to 650,000 individuals still lose their lives to flu-related complications annually around the world. Although the annual flu vaccine has undoubtedly saved countless lives, there is an ongoing challenge with consistently high efficacy, and many wonder what future strategies might enhance this protection, especially in preparation for the next pandemic threat.
A new body of research, published in the journal Science, sheds light on a factor that could be a game-changer for how we approach flu vaccinations: genetics. This study analyzed sets of twins and found that a significant portion of the immune response to seasonal flu vaccination is influenced by genetic makeup. On the surface, this discovery might seem straightforward—our genes are fundamental to nearly everything about us—but its implications for vaccine design are groundbreaking. Instead of being destined to mount an immune response set in stone by whichever viral strain we first encountered, there appears to be room for manipulation. In other words, the immune system can be encouraged to respond more flexibly to new or multiple influenza strains than previously assumed.
Central to this exploration is the dismantling of an idea known as “original antigenic sin,” frequently referred to by researchers with the shorthand OAS. In past decades, many scientists believed that the earliest flu strain a person encountered during childhood (be it a natural infection or a vaccine) shaped the pattern of that individual’s immune response for life. Once the immune system “learned” a particular subtype, it would hone in on that type each time it encountered a flu vaccination, ignoring the others included in the shot. This phenomenon was held up as an explanation for why a single individual might react robustly to one strain in a vaccine but seem almost indifferent to others, leaving large gaps in protection. While the OAS concept was influential, it left a major question unanswered: if original antigenic sin was so powerful, was there any way to override it and guide the immune system to mount a broader, more inclusive response?
Researchers at Stanford University, led by Mark Davis, PhD, discovered that the situation is more nuanced than OAS would suggest. By looking closely at identical twins and analyzing their responses to flu vaccination, the team noticed that prior exposure to certain viral subtypes played a role in shaping antibodies. Yet, interestingly, genetics emerged as a more powerful factor than simply the first strain encountered. This insight hinges on polymorphisms in the major histocompatibility complex (MHC) class II region of the genome. MHC class II molecules are crucial for presenting viral antigens to helper T cells, which in turn support B cells for antibody production. So, if a person’s genetic makeup encodes a particular set of MHC class II variants, this can dictate which parts of the virus get recognized most effectively—and thus which strain they might be biased toward fighting off.
This discovery opens the door to potentially overcoming subtype bias. The Davis team took this concept into the lab by designing a novel vaccine approach that includes multiple influenza subtypes tethered together on a molecular scaffold. Traditionally, flu vaccines incorporate four different versions of the hemagglutinin (the “hook” the virus uses to attach to cells). However, simply mixing these four antigens in a shot doesn’t guarantee that a recipient’s immune system will respond equally to all of them. Often, individuals demonstrate a robust response to just one or two subtypes, leaving them underprotected against others. The experimental approach from this study, however, couples the antigens in a specific way so that the body effectively “sees” them all simultaneously. In mouse models and tonsil organoids (derived from human tonsil tissue), this multi-subtype scaffold triggered broader T cell help, which translated into a wider range of antibody responses.
By expanding helper T cell diversity, a vaccine could counteract the genetic predisposition some individuals have to focusing on only one subtype. It’s as though the vaccine “forces” the immune system to recognize each included strain instead of letting it revert to old preferences. The authors of this research find this particularly exciting in the context of a future pandemic, which could be caused by an avian influenza strain (often referred to as bird flu). Since bird flu is of particular concern due to its high mortality rate in cases where it infects humans, any vaccine strategy that expands cross-strain immunity would be a powerful defensive asset.
The structure of the flu virus offers an interesting glimpse into why the virus is so elusive. Hemagglutinin, the protein used by influenza to latch onto cells in the respiratory tract, can vary dramatically among subtypes. A single seasonal vaccine contains antigens for four predicted strains (often a mix of influenza A subtypes like H1N1 or H3N2 and influenza B lineages). The problem is that this guesswork only works so well. If people don’t mount an immune response to each of those four strains, the vaccine’s overall efficacy drops. This partly explains why vaccine effectiveness can vary from year to year, often ranging somewhere between 40% and 60% in the best of scenarios. By introducing genetic insights into how people respond to vaccines, researchers can better customize or design next-generation immunizations to ensure a more uniform uptake of all intended targets.
One reason why the twin studies are particularly compelling is that twins, especially identical twins, share virtually the same genetic background. Any differences in their immune responses may be easier to link to environmental or other random factors. When the Stanford team noted that identical twins’ antibody responses were very similar, despite potentially different past exposures, it raised a red flag about the long-standing emphasis on OAS. It suggested that the inclination to respond well to certain flu subtypes and less to others might be rooted more in their shared genes than in whichever strains they might have first encountered during childhood. Further investigations in newborns, who had less diverse exposure to prior infections, reinforced this conclusion.
It’s worth underscoring that these revelations do not wholly dismiss original antigenic sin. The concept has a historical basis in real-life observations, and prior exposure to certain pathogens does shape immune memory. However, the findings imply that original antigenic sin might not be the sole determinant of how a person’s immune system responds when given a flu shot containing multiple strains. In other words, one’s first viral encounter might set the stage, but genetics writes a big part of the script.
By leveraging the power of modern vaccine design, scientists hope to exploit the flexible potential revealed by these experiments. T cells are a key player here. To produce a strong and broad immune response, you need a robust T cell response that recognizes multiple subtypes. These T cells then assist B cells in making antibodies that mark or neutralize the virus. If your genetics gear you to see only H1N1, for example, a regular shot might not be enough to alert your immune system to H3N2, H5N1, or B strains in the vaccine. By coupling multiple subtypes in a special way, or by engineering a scaffold, vaccine designers can circumvent that genetic predisposition and effectively “trick” the immune system into paying attention to all the included subtypes.
This approach offers an appealing avenue for preparing against a looming threat: the next pandemic strain. In the past century, influenza pandemics occurred in 1918 (the Spanish flu), 1957 (the Asian flu), 1968 (the Hong Kong flu), and 2009 (the H1N1 swine flu). Each pandemic emerged when a drastically different strain of the influenza virus began infecting humans, typically one that many immune systems had never seen before. Bird flu strains, such as H5N1 or H7N9, have already caused severe disease in individuals who had direct contact with infected poultry. Fortunately, these strains don’t often spread efficiently between people—yet. Should the virus mutate to become more easily transmissible, we could face another pandemic on a global scale. The ability to produce broader, cross-reactive antibodies through novel vaccine designs might be one of the best hopes of mitigating that threat.
When it comes to implementing new methods, though, vaccine design is only part of the puzzle. Scientists must also consider regulatory pathways, large-scale manufacturing, distribution logistics, and public acceptance. Even the most groundbreaking vaccine will fall short of its potential if only a small fraction of the population chooses to get vaccinated. Moreover, as the flu virus continues to mutate over time, ongoing research will likely be necessary to update vaccine formulations so that they remain effective against the latest circulating strains. Still, embedding broader reactivity within the design from the get-go could help maintain at least partial coverage, even against newly emerging variants.
These findings also illustrate a broader principle relevant to many infectious diseases: the interplay between environment and genetics is almost never a simple either/or proposition. It’s both. Historically, if a vaccine faltered, the blame might have been placed squarely on the virus’s rapid evolution or on an individual’s prior exposures. Now, it appears that certain genetic elements can significantly predispose a person’s immune system to react in specific ways. This is a critical piece of the puzzle that could help scientists craft not only better flu vaccines but also improved vaccines for diseases like HIV, malaria, or coronaviruses that have multiple strains or rapidly changing subtypes.
As for the molecular scaffold approach mentioned in the study, it’s a versatile strategy. The research team successfully showed that by using a matrix to physically link four different hemagglutinin variants, mice and human tonsil organoid models developed a stronger immune response to all four. This technique can be adapted for other pathogens, as noted by the investigators. Anywhere that multiple strains or subtypes exist simultaneously, a coupling strategy to broaden T cell help might yield a more protective immune profile. In concept, if new subtypes appear in subsequent seasons, this flexible design could continue to incorporate them. In theory, you might even design a single vaccine that accounts for multiple lines of influenza A, B, and other emergent strains, helping people avoid the mismatch problem altogether.
Another key insight is how the study used tonsil organoids. While many people think of tonsils only when they’re dealing with a sore throat or a recommended tonsillectomy, these tissues are part of the immune system’s first line of defense in the throat region. By using organoids cultured from human tonsil cells, scientists can gain a close approximation of how an actual human immune system might respond to a new vaccine design, without exposing volunteers to untested formulations in early research. This speeds up development and refines the process for when vaccines enter clinical trials.
The team’s approach is particularly exciting for addressing the challenge posed by bird flu, which Mark Davis highlights as a likely candidate for the next pandemic. Viral strains like H5N1 and H7N9 can cause very severe illness. In cases where they jump from birds to humans, fatality rates can be alarmingly high, though sustained human-to-human transmission remains rare. Nevertheless, all it takes is one or two critical mutations for that reality to change. Should a strain emerge that spreads efficiently among humans, it could lead to a global crisis. Preemptively formulating a vaccine that accounts for multiple subtypes, including those found in birds, could buy time and save lives if a sudden outbreak of a pandemic-capable strain were to occur.
Public health authorities have long recognized the necessity of anticipating which strains of flu are most likely to circulate during the upcoming season. Each year, the World Health Organization and other bodies review global data, attempt to predict the dominant strains, and pharmaceutical companies manufacture large quantities of vaccine based on that guess. While often on target, it’s far from a perfect system. A shift known as “antigenic drift” can alter the virus just enough to render the vaccine less effective. Rarely, a more drastic change called “antigenic shift” can lead to a completely new subtype. The possibility of bridging those gaps with a more flexible, genetically informed vaccine is a major step forward.
From a real-world standpoint, seeing this science move from the lab bench to clinical application will be the ultimate test. Researchers must demonstrate safety and efficacy in larger human trials, produce the vaccine at scale, and work through the regulatory checks that ensure high standards of quality. If successful, the benefits could extend far beyond typical seasonal flu prevention. It might reshape how we handle pandemic preparedness by giving public health experts a broader, longer-lasting tool against unpredictable viral changes. This same principle could potentially be applied to other infections that frequently mutate or have multiple subtypes.
Looking back at the bigger picture, there’s also a takeaway about how valuable it is to question longstanding assumptions in science. Original antigenic sin was first proposed decades ago, backed by compelling observational evidence. Yet as technology evolves, particularly in immunogenetics, it becomes possible to take a deeper look at complex phenomena. When scientists discovered that genetics can trump or at least rival the importance of initial viral exposure, it demonstrated that our immune systems are more adaptable than we once believed. This adaptability is a double-edged sword, in that the virus continues to adapt as well, but every new piece of evidence on how to steer the immune response is a win for public health.
It is often said that the perfect flu vaccine is a “holy grail” of immunology—something that could provide life-long protection against any strain, effectively rendering seasonal shots obsolete. While that remains elusive, each incremental advance moves us closer to the day when we can protect people on a broader and more predictable scale. These new genetic findings and the innovative vaccine design described in the Science publication highlight a plausible path toward that goal. By coupling multiple antigens, engaging the right T cells, and respecting each individual’s genetic predispositions, flu vaccines could be far more versatile than they are now.
Such a development could not come at a better time. In today’s interconnected world, viruses have greater opportunities to travel quickly across regions and continents. A pathogen that emerges in one area can reach distant populations in just a few days. During the 2009 swine flu pandemic, it took mere months for the virus to spread worldwide. Public health preparedness requires advanced vaccines that can respond to these threats. The notion that an immune system is “locked” into a single response is not only discouraging but also outmoded by recent data. We now have more evidence that with the right approach, the immune system can be guided to mount strong, multi-pronged defenses.
In the event of a future pandemic, whether it is a flu strain or another type of virus, the lessons learned here will apply. The path to pandemic preparedness involves understanding how genes, environment, and pathogen features interact, and designing vaccines accordingly. As our comprehension of MHC class II polymorphisms and other genetic factors deepens, personalized or semi-personalized vaccines might even become a reality in the more distant future. In the nearer term, coupling antigens to broaden T cell help is already a major leap forward, with implications for diseases beyond influenza.
It’s also crucial to maintain robust surveillance of human and animal populations for new flu strains. If a strain like H5N1 mutates and starts spreading efficiently, scientists will have a head start if they already have a scaffolded vaccine template that can be quickly adapted. The capacity to plug in new antigens to a proven platform, rather than building an entirely new vaccine from scratch, can dramatically cut down on lead times. This might be the deciding factor in preventing a localized outbreak from becoming a global catastrophe.
In the grand scheme, flu is still a leading cause of vaccine-preventable death, and every piece of progress in this field has the potential to save countless lives. Understanding how genetics shapes response, and how to broaden that response, adds another layer of sophistication. Although we are not yet at the finish line for a universal flu vaccine, the work referenced here offers a promising step in that direction, illustrating how science continually refines our strategies for battling one of humanity’s most persistent viral foes.